Neutron and Gamma Ray Imagers
Our Legacy Imagers
Many of developments in radiation imaging date back over 30 years to our involvement with Nuclear Medicine in the development of instrumentation for gamma-ray imaging. Since the medical instrumentation industry represents one of the largest commercial outlets for radiation instrumentation, we expect that our present involvement in this area will continue to grow in the foreseeable future. The camera shown in the figure above is our first radiation imager applied for environmental imaging, and the success initiated generations of more sophisticated and complex radiation imagers. The radioactive materials were produced in our nuclear reactor, and the images shown required 1 graduate-student-honeymoon, acquired remotely. That was quite a feat in 1993.
After exploring simple mechanical collimators to form images, we led the development of Compton scattering techniques that avoid the efficiency loss due to the collimator in conventional gamma-ray cameras. While there appears to be fundamental limits in the use of this technology at the low gamma ray energies currently predominate in nuclear medicine studies, we recognized there would be decided advantages in their application to higher energy gamma rays in either a medical or industrial context. Currently, there is a great deal of interest in techniques for higher energy gamma ray imaging for use in the decontamination of nuclear facilities or in fast neutron imaging for the detection of clandestine nuclear materials. For these reasons, imaging studies have become an even more active research field.
Over the past three decades, we have developed families of mechanically-collimated (e.g., parallel-hole collimator, coded-aperture) and electronically-collimated (i.e., Compton) radiation imagers. We then moved into hybrid cameras that utilized both types of imaging in a single instrument (e.g., SORDS) More recently, we have been engaged in developing portable dual-particle imagers (to detector both gamma rays and fast neutrons for nonproliferation applications) using time-encoding. These devices have the advantage of simplicity and cost-effectiveness for field applications.
Time-Encoded Imaging
Time-encoded imaging (TEI) was invented at Michigan for nuclear medicine imaging. The technique has the advantage of encoding source radiation through a time-varying mask pattern on a single detector rather than encoding the source radiation through a fixed mask in space on an array of detectors. The advantage of the TEI technique is simplicity (it requires only a single radiation detector) and cost, while the disadvantage is typically a longer acquisition time. When multiple imaging systems are desired, the TEI advantages may prove dominant.
Time-Encoded Gamma Ray and Fast Neutron Imaging with an Asymmetric Mask (MATADOR)
The MATADOR imaging system is a 1D, dual-particle, adaptive cylindrical TEI system. MATADOR utilizes a dual-layer mask and two non-position sensitive detectors to image both gamma rays and fast neutrons. To modulate gamma rays, the inner layer of the mask is made of 0.635 cm of tungsten, and to modulate fast neutrons, the outer layer is made of 6 cm of high-density polyethylene. Both mask layers are arranged in a uniformly redundant array pattern with 35 elements, thus the angular width of an element is ∼10.3◦. The outer radius of the mask is 25.7 cm and MATADOR can collect a full revolution of data in 90 s.
To detect both particles, MATADOR utilizes two detectors: a 1” Cs2LiLa(Br, Cl)6 (CLLBC) detector to detect gamma rays and a 2” stilbene detector to detect fast neutrons. One could also use the gamma rays detected by the stilbene detector to reconstruct gamma-ray images but the lack of photopeaks would increase background. Thus, we use the CLLBC data for gamma-ray reconstructions.
MATADOR has the additional capability of moving the detector within the rotating mask assembly, allowing for different fields of view. To demonstrate the value of adaptive detector movements in a complex scenario, we set up a multiple source experiment at the ZPPR facility at INL using special nuclear material. The setup includes 5 radioactive objects made using metallic plutonium plates with a high concentration of Pu-240 and mixed oxide (MOX) fuel pins.
They are of varying intensity at different radial distances from the system. The figure shows a picture of the setup and the reconstructed results using adaptive movements. Notice that the two sources at 90◦ cannot be resolved when the detector is at the center, but when we utilize data from off-center detector positions, the two sources are clearly resolved. Thus, even in a challenging scenario with significantly stronger sources in the FOV, repositioning the detector improves angular resolution. One should note though that the performance for sources significantly off-axis from the imaging axis, such as the object at 200◦, may be worse in the adaptive case than the conventional. Thus, the user must decide which objects are important to capitalize on the source localization and angular resolution advantages.
In spite of these limitations, adaptive detector movements have the potential to improve the localization precision and angular resolution of a c-TEI system without increasing the mask radius or reducing the detector size. The improved performance also translates to a shorter time-to-image. Sinclair et al. define time-to-image for a gamma-ray imager as the measurement time required to achieve a root-mean-squared error (RMSE) of 2◦ for a 1 mCi Cs-137 placed 1000 cm from the system. To find the time-to-image for a fast neutron imager, we use a similar definition except the source is a 1 mCi Cf-252 source instead of a 1 mCi Cs-137 source. We simulated Poisson replicates of the expected observation vector as a function of measurement time and estimated the source position using ML. We assume that one, full revolution of data is collected with a S:B ratio of 2.2:1.
When the detector is centered, the time-to-image is 110.0 s, and when the detector is at the optimal detector position, the time-to-image is 100.3 s, which is a reduction of 8.8%. Notice though that if time-to-image is defined differently, the reduction in the time-to-image changes. For example, the time to reach a RMSE of 0.7◦ is 266.8 s in the centered case and 127.0 s in the off-center detector case, which is a substantial improvement of 54.4%. The reduction in the time-to-image is relatively constant for RMSEs below 0.7◦ and approaches the asymptotic improvement factor predicted by the Cramer-Rao Lower Bound, which is 56.4%.
These benefits from adaptive detector movements come at a relatively small increase in cost and complexity. The addition of an x-y linear stage to move the detector is straightforward and one can imagine a simple adaptive algorithm, where the system collects data with the detector at the center for a predefined period of time, requests user input to define regions-of-interest, and then repositions the detector accordingly. The low cost and complexity of adaptive detector movements make them an attractive option to improve the localization precision and angular resolution of TEI systems.
The lessons learned from MATADOR have led to its successor, LANTERN. LANTERN is being designed to be much smaller and lighter in order to trade some angular resolution for mobility. Different mask designs are being considered, such as hollow or variable density printed masks.
The RMC: A rotating modulation imager for locating mid-range point sources
Rotating modulation collimators (RMC) are relatively simple indirect imaging devices that have proven useful in gamma ray astronomy (far field) and have more recently been studied for medical imaging (very near field). At the University of Michigan a RMC has been built to study the performance for homeland security applications.
One class of time modulated systems is the rotating modulation collimator (RMC). One of the most appealing characteristics of this particular design is that they do not require position sensitive detectors in order to locate sources of interest. This feature allows for a significant amount of flexibility in not only detector material selection, but also in the geometry and size of the detectors employed. It also offers a simple way to enhance the current network of non-position sensitive detectors by offering a point source imaging system that snaps on to the front of common detectors like the ORTEC DETECTIVE. The focus of this work was on a RMC system that has been developed for locating hidden point sources in a mid-range (1–50 m) field. One of the motivating factors for using RMCs is their ability to achieve excellent angular position resolution over a relatively wide field of view (FOV).
In the case of a RMC system, a set of two or more attenuating masks are separated by a known distance as shown in the figure. One typical pattern for the masks is straight parallel slits made of tungsten or lead for gamma ray imaging. When the masks are rotated together, the photons counted by the detector from any source that is off of the centerline axis will be modulated as the slits appear to the source to open and close producing a unique pattern. Both the frequency of the peaks as well as the phase of the pronounced dip contains the information necessary to locate the source by applying an appropriate reconstruction algorithm.
Two-dimensional mapping of the system was accomplished using a Ba-133 source located 215 cm from the front mask of the detector. The distance between the masks was fixed at L = 24 cm. The source was placed on a two axis Velmex bi-slide stepper motor system that allows the source to translate in both the x- and y-dimensions with sub-millimeter accuracy.
Measurements with the prototype RMC in the active sensing region, show that position estimation using the ML-EM algorithm is very accurate. With the exception of the source located at r = 0, the average standard deviation on the position estimate for sources located between the blind zone and field of view edge is 1.6 arc minutes.
The performance of RMCs had been already demonstrated for both astronomical and medical imaging. From the data acquired, RMCs represent a practical way to locate point sources of interest to the homeland security community using any single non-position sensitive detector. Continued research should focus on the relationship between the detector energy resolution and RMC angular resolution, the effects of mask penetrations on images and potential correction factors, the effects of multiple and extended source in the field of view, and adaptive imaging techniques for changing the RMC sampling profile during a measurement to locate sources faster.
Future Directions
Our past work used cylindrical geometry to provide a convenient, fixed rotation axis. A more ambitious goal is to move to spherical geometry. Imagine a rolling robot with an outer shell that serves as the mask that projects the radioactive environment on a centered radiation detector. The “Sphero” BB-8 robot is commercially available and driven by a phone app. Extending the hardware to larger geometry seems reasonable and incorporating a small scintillator with a SiPMT would provide the detector. The geometry of the mask pattern still needs to be thought about.
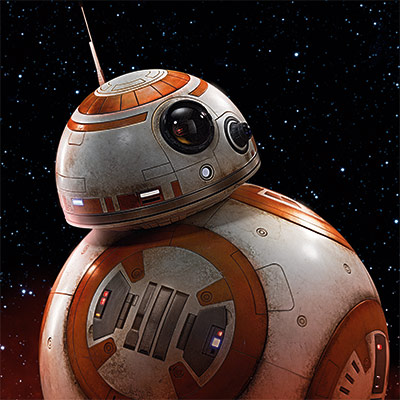
Recent work in this area can be found: